Ocean oxygenation and the global carbon budget
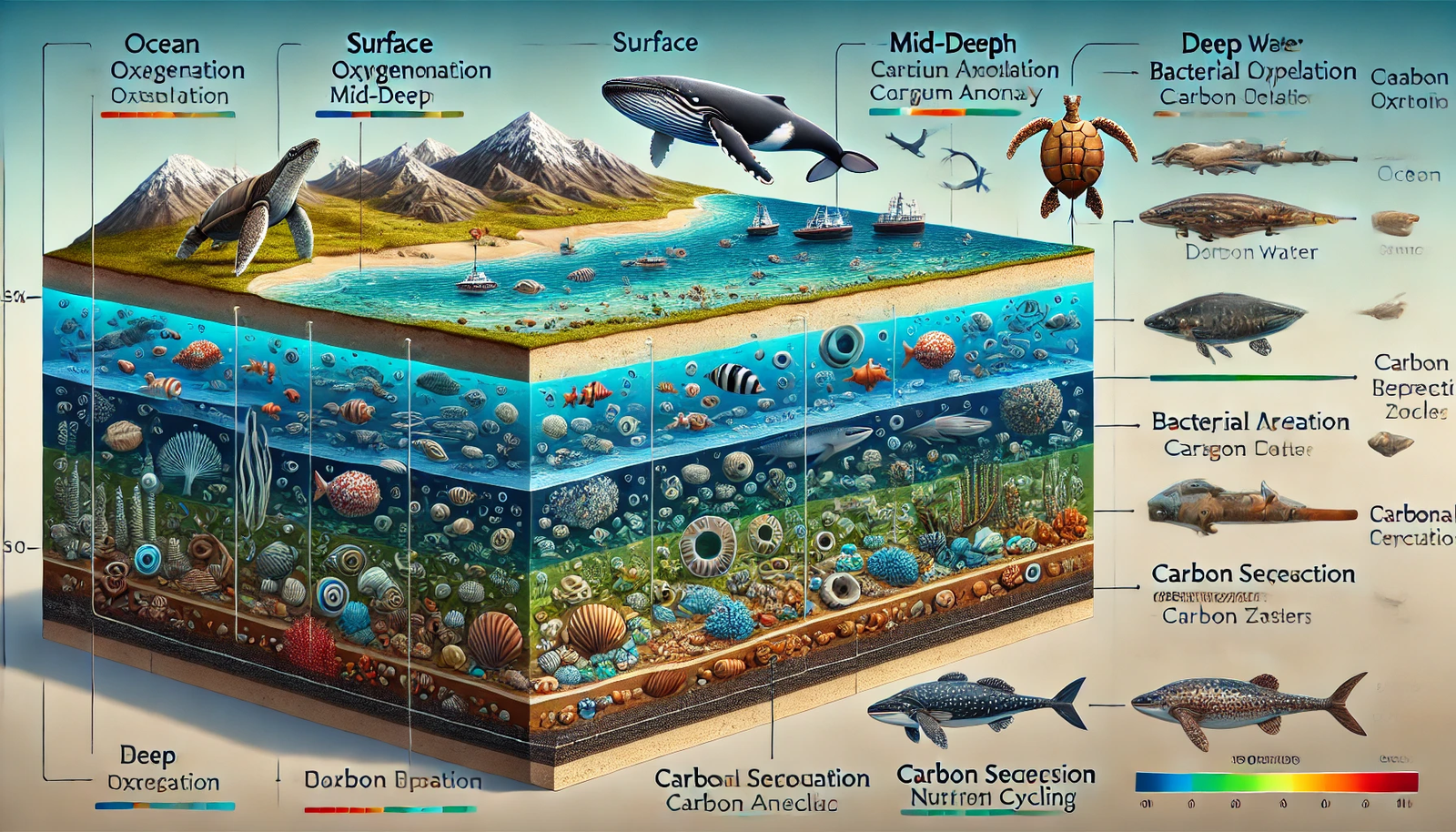
Ocean oxygenation is indeed a critical factor in controlling the global carbon budget and influencing major nutrient cycles due to its tight coupling with carbon and oxygen dynamics. As bacterial respiration consumes oxygen to break down organic matter generated by photosynthesis, this process simultaneously releases carbon back into the water column. The resulting accumulation of respired carbon directly impacts oxygen levels and nutrient availability, which in turn regulate primary productivity and carbon sequestration in the ocean.
Key Aspects for Investigation:
- Link Between Oxygen Levels and Carbon Cycling:
- The rate of bacterial respiration directly influences how much oxygen is removed from the water column, with more intense respiration leading to lower oxygen levels and increased carbon accumulation. This relationship is key to understanding how ocean oxygenation can regulate the carbon budget.
- Implications for Major Nutrient Cycles:
- Reduced oxygen levels, or hypoxia, affect nutrient cycling, especially for elements like nitrogen and phosphorus. In low-oxygen conditions, denitrification processes dominate, reducing nitrate levels, while phosphorus can be released from sediments, altering the nutrient balance and availability for marine life.
- The Role of Redox-Sensitive Elements (like Cerium):
- Redox-sensitive elements, including Ce, provide clues about oxygenation conditions in the water column. For instance, Ce(III) oxidation to Ce(IV) in oxygen-rich environments and subsequent removal via scavenging creates a “Ce anomaly,” which can serve as a proxy for past and present redox conditions.
- Microbial vs. Abiotic Processes:
- The finding that microbial activity primarily drives Ce(III) oxidation suggests that microbes play a key role in shaping the redox landscape of the ocean. Understanding the extent of microbial versus abiotic redox reactions could clarify the mechanisms behind the accumulation or removal of specific elements, like Ce, in response to changing oxygen levels.
- Impact of Surface Oxygen Maxima and Light Inhibition:
- Sunlight inhibition of microbial oxidation and scavenging at the ocean’s surface leads to the characteristic surface maxima of elements like Ce and Mn. Investigating the role of sunlight in regulating these redox processes could enhance our understanding of element distribution patterns and how they correlate with oxygenation.
- Potential Indicators of Ocean Oxygenation:
- By measuring anomalies in Ce and other redox-sensitive elements across different depths and regions, we can create a profile of oxygenation patterns. Coupling these measurements with carbon and nutrient analyses can further reveal how oxygen levels influence broader oceanic cycles.
Methods of Investigation:
1. Comprehensive Sampling and Analysis:
- Seawater and Sediment Collection: Obtain seawater and sediment samples from various depths (surface to deep) in regions like hypoxic zones, oxygen minimum zones, and carbonate-rich environments.
- Shell and Carbonate Rock Sampling: Collect shells and carbonate rocks from recent and ancient deposits to study past and present oxygenation conditions.
2. Trace Element and Isotope Analysis:
Trace elements are dynamic in marine environments due to their interaction with biological, chemical, and physical processes.
- Biological Cycling: Many trace elements (e.g., iron, zinc, copper) are crucial for marine organisms, as they serve as cofactors for enzymes and are involved in cellular processes like photosynthesis and respiration. Marine life uptakes these elements from seawater, and upon decomposition, these elements can be released back into the water column or settle as part of organic debris on the seafloor.
- Chemical Interactions: Trace elements like manganese and iron undergo redox transformations that influence their solubility and mobility. In oxygen-rich water, iron and manganese tend to form oxides that can precipitate and settle to the seafloor. In contrast, under low-oxygen or anoxic conditions, these elements can be more soluble and move through the water column more freely.
- Sediment Accumulation: On the seafloor, trace elements become part of the sediment through various pathways:
- Direct Precipitation: Some elements precipitate as minerals, especially in reducing environments, leading to enrichment in certain sediments.
- Adsorption: Trace elements can adsorb onto particles like clay minerals, organic matter, or iron/manganese oxides, enhancing their accumulation in sediment layers.
- Biogenic Sediment Incorporation: Elements taken up by marine organisms can be incorporated into biogenic sediments (like shells), locking them into the rock record.
Studying these trace element enrichments, especially in fossil shells or sedimentary layers, offers insights into past seawater chemistry, nutrient cycling, and even broader environmental shifts like ocean anoxia events or changes in marine productivity.
Redox-sensitive trace elements such as uranium (U), molybdenum (Mo), vanadium (V), and cerium (Ce) are excellent proxies for reconstructing past environmental conditions because they are sensitive to oxygen levels and biological cycling in marine systems. Their distribution in sedimentary rocks can reveal crucial insights into historical redox states and biogeochemical processes.
Here is how they function as proxies:
- Uranium (U): Uranium is more soluble under oxic conditions and tends to precipitate as U(IV) under reducing conditions. Elevated U concentrations in sediments often indicate low-oxygen or anoxic environments. U isotopes can also provide additional detail, helping to distinguish between various anoxic conditions, such as euxinic (sulfide-rich) versus ferruginous (iron-rich) environments.
- Molybdenum (Mo): Molybdenum is highly enriched in euxinic (anoxic and sulfide-rich) environments. Because it requires both low oxygen and high sulfide for its enrichment, high Mo concentrations can indicate strongly reducing conditions and the presence of sulfide in pore waters or overlying seawater.
- Vanadium (V): Vanadium exhibits complex redox behaviour, existing in multiple oxidation states. V is typically enriched in organic-rich sediments and is associated with anoxic environments, but it can also occur in ferruginous settings. V/Cr ratios are often used to assess the intensity of anoxia, with higher ratios suggesting more reducing conditions.
- Cerium (Ce): Cerium’s unique behaviour in seawater, where it can be oxidized and removed from solution in oxic waters, leads to a distinct “Ce anomaly” in REE patterns. A negative Ce anomaly often indicates an oxic water column, while the absence of a Ce anomaly may suggest reducing conditions or limited oxygenation.
By studying the distribution and ratios of these elements, you can track significant changes in marine redox conditions over geologic timescales, such as shifts linked to mass extinctions, ocean anoxic events, or the evolution of marine ecosystems. Additionally, coupling these proxies with other isotope systems (e.g., Sr, Nd, or Ca isotopes) can provide a more comprehensive picture of past ocean chemistry and paleoenvironmental conditions.
3. Radiotracer and Isotopic Studies:
- Radiotracer Experiments: Use radiotracers for Ce(III) oxidation studies to monitor redox reactions in real-time, and assess the role of microbial versus abiotic oxidation.
- Stable Isotope Ratios: Measure stable isotope ratios (e.g., δ⁴⁴Ca, δ⁸⁸Sr, δ⁶⁶Zn) in shells and carbonates to interpret biological processes, diagenetic changes, and environmental conditions.
- Calcium (Ca) Isotopes: Reflect metabolic and environmental conditions, potentially linked to productivity and the carbonate cycle.
- Strontium (Sr) Isotopes: Use Sr isotopic signatures to study seawater composition, particularly for paleoceanography. Sr isotopes are indicators of weathering and hydrothermal inputs, which are influenced by ocean oxygenation.
- Zinc (Zn) Isotopes: Zn isotopes are redox-sensitive and can reveal information about biological activity and nutrient cycling, as Zn is often taken up by phytoplankton.
4. Shell Analysis as Indicators of Past Conditions:
- Trace Elements in Shells: Use shell chemistry (REY, Ca, Sr, Zn, Ta) to trace variations in ocean oxygenation and nutrient availability. Ce anomalies and isotopic variations in shells can indicate fluctuations in redox conditions, helping us understand ancient oceanic events like anoxic events.
- Paleoenvironmental Reconstruction: Combine isotopic and trace element data from fossil shells and carbonate rocks to reconstruct historical oxygenation levels and carbon sequestration dynamics, correlating findings with known global events (e.g., mass extinctions, glacial-interglacial cycles).
This investigation will deepen our understanding of how trace elements and isotopes respond to redox changes, providing tools for reconstructing past ocean oxygenation and nutrient cycling. By examining shells and carbonate rocks, we can gain valuable insights into ancient oceanic conditions, crucial for interpreting Earth’s climate and biogeochemical cycles over time.